When LIGO, the Laser Interferometer Gravitational-Wave Observatory, first detected gravitational waves from merging black holes, it opened up a new window in astrophysics and provided the most powerful confirmation yet of Einstein’s theory of general relativity. Now LIGO has done it again, together with the Virgo interferometer, this time by observing merging neutron stars – something astrophysicists had known must happen but had never been able to detect definitively until now.
Observing two neutron stars smash together is important for much more than just the thrill of discovery. This news may confirm a longstanding theory: that some gamma-ray bursts (GRBs for short), which are among the most energetic, luminous events in the universe, are the result of merging neutron stars. And it is in the crucible of these mergers that most heavy elements may be forged. Researchers can’t produce anything like the temperatures or pressures of neutron stars in a laboratory, so observation of these exotic objects provides a way to test what happens to matter at such extremes.
Astronomers are excited because for the first time they have gravitational waves and light signals stemming from the same event. These truly independent measurements are separate avenues that together add to the physical understanding of the neutron star merger.
Gravitational waves just one part of this news
The LIGO project has thus far announced the detection of four mergers of binary black holes – observed via the gravitational waves they emitted. These are ripples in the fabric of spacetime propagating in all directions, like waves emanating out from a pebble dropped in a pond. Encoded in the gravitational wave signal is information about the pre- and post-merger masses of the objects. Black holes are much more massive than neutron stars, so the energy they release as gravitational waves is much higher. Because light cannot escape from a black hole, you expect (and see) no light from these mergers.
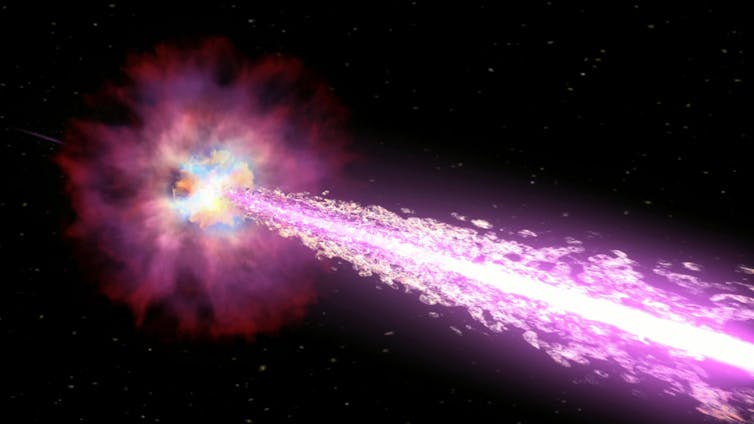
NASA/Swift/Cruz deWilde, CC BY
The merger of neutron stars should produce both a gravitational wave and a short gamma-ray burst signal. These brief, incredibly intense flashes of gamma-ray light are seen from galaxies across the universe. They come in two types, classified by their duration. Short GRBs are thought to come from the mergers of neutron stars, while long GRBs are known to be coincident with supernovas.
Key to unlocking the mystery of any astronomical object is knowing its distance. In recent years, astronomers have identified the host galaxies of a handful of short GRBs. Determining those galaxies’ distances allows astronomers to calculate the power emitted in gamma-rays during the burst, and to determine (or rule out) physical scenarios that could produce that power.
But for LIGO to detect two neutron stars spiraling in toward each other and merging, it would need to happen relatively nearby – within around 250 million light-years. That such an event was not detected during the first year and a half of LIGO observations already lets astronomers place a constraint on how frequently they happen in the nearby universe.
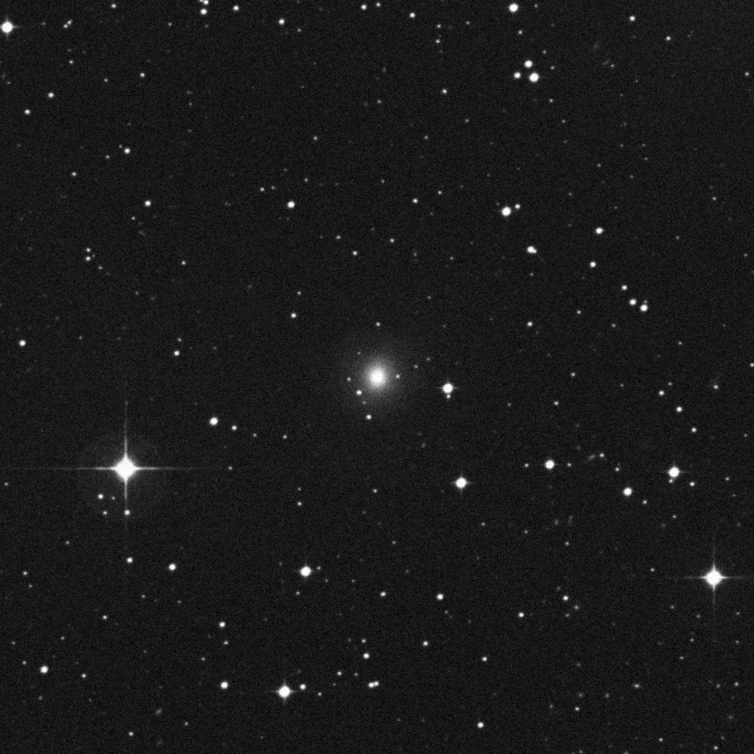
Palomar Observatory – Space Telescope Science Institute Digital Sky Survey, CC BY
So the rumor of a merging neutron star detection by LIGO with a coincident short gamma-ray burst (GRB170817A) seen by NASA’s Fermi Gamma-ray Space Telescope spread through the astronomical community like wildfire this past summer. Astronomers watched from the sidelines as most of the major telescopes in (and above) the world slewed toward an otherwise unremarkable old, nearby (130 million light-years) elliptical galaxy named NGC 4993.
What we’ve known about neutron stars
Most stars end their lives relatively calmly; no longer supported by the fusion of hydrogen into helium, their outer layers glide slowly off into space while their cores collapse to the very limits allowed by normal matter – burning embers the size of the Earth called white dwarf stars.
For the rare stars whose masses are a bit higher, 10 to 20 times that of the sun, the picture is a bit different. These stars die the way they lived: quickly and violently, ejecting their outer layers as supernovas and leaving behind something far stranger – a neutron star.
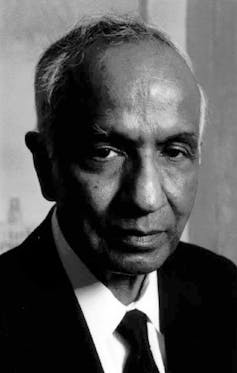
AP Photo
The details of this story were worked out in 1930 by then 19-year-old Indian astrophysicist Subrahmanyan Chandrasekhar. He determined precisely how far you can compress normal matter before the relentless pressure of gravity forces electrons into the nuclei of their atoms where they merge with protons to form neutrons. Instead of an Earth-sized remnant, a massive star’s core collapses further to become a highly compressed ball of exotic matter as small as a city but whose mass can be twice that of the sun.
Neutron stars rotate incredibly rapidly. The collapse from millions to tens of kilometers in extent increases their spin due to conservation of angular momentum, like an ice skater pulling in her arms. While the parent star may have rotated once a month, a newly born neutron star can spin hundreds of times per second.
This rapid spinning led to their initial discovery. 50 years ago, Antony Hewish and Jocelyn Bell Burnell discovered the first radio pulsar: a neutron star emitting radio waves which appear to observers as pulses as the star rotates, like a lighthouse. Hewish would win the 1974 Nobel Prize in physics for this discovery, while Bell Burnell was controversially overlooked.
But what are neutron stars really made of? Are they neutrons all the way through or can they break down further again, into what physicists call “quark soup”? The answer lies in measuring their size. A larger neutron star is mostly neutrons, a smaller star has a more complicated interior made of quarks – the building blocks of protons and neutrons. Untangling how this works is important for our understanding of the fundamental properties of subatomic particles. A new telescope on the International Space Station aims to address this question by targeting neutron stars and measuring their sizes.
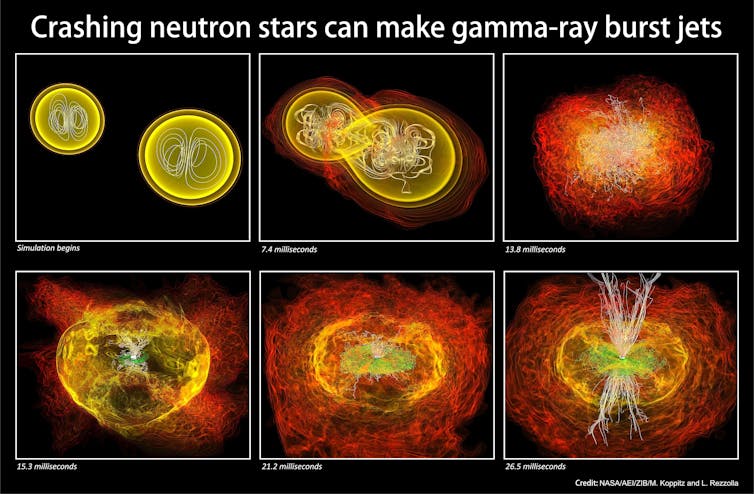
NASA/AEI/ZIB/M. Koppitz and L. Rezzolla, CC BY
When neutron stars merge
Over half of all stars are part of binary pairs, and massive stars are more likely to occur in binaries. These pairs of massive stars will co-evolve, and when they die, a pair of neutron stars may remain, orbiting one another.
An orbiting pair of neutron stars loses energy by emitting gravitational waves, and over time this loss of energy will cause them to migrate closer and closer until they eventually collide. While the eventual merger is nearly instantaneous, the gradual inspiral takes tens to hundreds of millions of years, so we expect to see mergers in more evolved galaxies – like NGC 4993, for instance – rather than those that are still rapidly forming new stars.
For decades, it has been suggested that merging neutron stars may provide a mechanism for producing most of the elements on the periodic table heavier than iron. These so-called r-process elements must form in a neutron-rich environment, and have been formed by humans only during the explosion of nuclear bombs.
The signal from such an event is suspected to rapidly cascade through the electromagnetic spectrum, from gamma-rays to X-rays, visible light and infrared. Known as kilonovas, these afterglows have been seen from past short GRBs.
Finally all the pieces fall into place with this gravitational wave detected by the LIGO and Virgo teams, and all the subsequent supporting observations made by astronomers around the world. We know the neutron star masses, the duration of the event, and the distance of the host galaxy. This not only confirms the hypothesis that merging neutron stars produce short GRBs; it lays the foundation for astronomers to produce models of the merger backed both by fundamental physics and real world observations. It’s a rare event to see something new for the first time, and rarer still that it confirms a longstanding theory.
Roy Kilgard, Research Associate Professor of Astronomy, Wesleyan University
Shares